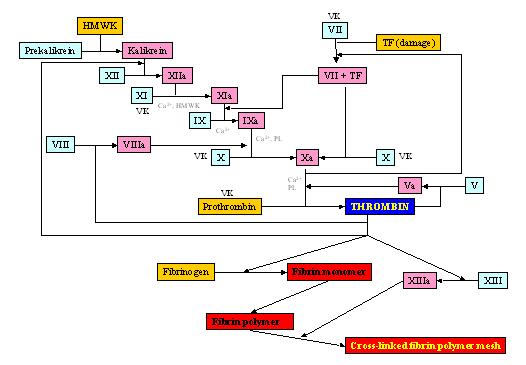
Figure 1. The proteolytic coagulation cascade of vertebrates. The vertebrate coagulation cascade is highly conserved among all vertebrate species. The cascade of proteolytic activations leads to the formation of an insoluble, fibrin meshwork. Several of the steps are dependent on divalent Ca2+ ions and phospholipids. Factors II (prothrombin), VII, X, and XI require Vitamin K as a co-activator.
Vertebrate clotting defects
Defects in or inhibitions of vital factors in the coagulation cascade lead to uncontrolled bleeding in cases of injury to the vasculature. Factors X and V Deficiencies and von Willebrand disease are examples of such bleeding diseases (Girolami et al., 1970; Hurtubise et al., 1979; von Willebrand, 1931) The hemophilias are characteristic genetic diseases that result in defects in various clotting factors.
Hemophilia A is caused by a defect in the gene that produces the blood clotting protein, Factor VIII. Several defects in the FVIII gene include inversions, deletions, insertions, frameshift and splice junction changes, polymorphisms, and nonsense and missense mutations. Windsor et al. (1995) observed 2 de novo factor VIII mutations, an X chromosome deletion, and a paternal FVIII inversion mutation. Antonarakis et al. (1985) found deletions of about 80 Kb in the factor VIII gene, and they also demonstrated a single nucleotide change in the coding region of the FVIII gene. Such a defect caused a nonsense mutation that led to the early termination of FVIII synthesis. Deletions of about 2000 bases in exon 3 were discovered by Higuchi et al. (1988). Two different point mutations, in exons 18 and 22, respectively, produced a nonsense codons by the substitution of CG to TG (Youssoufian et al. 1986). Kazazian et al. (1988) discovered L1 insertion elements (LINES) in exon 14 of the FVIII gene, and Woods-Samuels et al. (1989) observed a third L1 insertion in intron 10 of the FVIII gene. Bardoni et al. (1988), Youssoufian et al. (1988), and Brocker-Vriends et al. (1990) detected partial deletions of the FVIII gene in hemophilia patients.
Hemophilia B is characterized by a defect in Factor IX. Severe hemophilia B (also called Christmas disease) is caused by gross gene alterations, frameshift or splice junction changes, or nonsense or missense mutations; mild or moderately severe hemophilia B is predominantly associated with missense changes (Giannelli et al. 1992 , Thompson and Chen 1993 , Ketterling et al. 1999 , Weinmann et al. 1998). The original documented case of hemophilia B was that of a 16-year-old male named Stephen Christmas (1947-1992), and his FIX mutation (cysteine 206serine) was characterized by Taylor et al. (1992).
Hemophilia B Leyden is characterized by diagnosis of hemophilia B in youth with disappearance of the bleeding diathesis as the patient ages (Veltkamp et al. 1970). Before puberty, individuals with hemophilia B Leyden may suffer from severe hemophilia (<1% functioning FIX), but after puberty, the individual may seem normal with FIX functionality of 50% or greater (Briet et al. 1982). Reitsma et al. (1988) found that patients with hemophilia B Leyden had a single point mutation of T to A at position -20 of the FIX gene; this discovery led to the hypothesis that the disease is a result of a promoter mutation. Promoters are the areas of a gene that control the degree of expression of that gene by "turning it on or off." At birth, the FIX gene is turned off in hemophilia B Leyden patients. Reitsma et al (1988) also suggested that the point mutation they witnessed could lead to a switch from constitutive to steroid hormone-dependent gene expression. Thus, at puberty, the androgen (testosterone) produced by a young male can facilitate the expression of the FIX gene, and normal levels of active, functioning FIX protein can be produced.
THE INVERTEBRATE BLOOD COAGULATION SYSTEMS
Coagulation and immunity
Invertebrates lack an adaptive immune system but evolved an effective intrinsic defense against a variety of microbial pathogens (Kairies et al., 2001). All multi-cellular organisms, including humans, possess an innate immune defense, which suggests an evolutionary connection among them. The hemolymph of invertebrates has coagulatory properties that participate in both immune defense and hemostasis, but there is no contact activation mechanism. Coagulation of the hemolymph is only initiated as a response of the hemocytes to pathogen-associated molecular patterns (PAMP) (Ariki et al., 2003) such as intoxication by bacterial endotoxins like lipopolysaccharaid (LPS); this is in contrast to coagulation initiation in vertebrates, which is highly dependent upon endogenous factors (Theopold et al., 2004; Nakamura and Levin, 1982). When bacteria enter the hemocoel through an opening in the body of an invertebrate (i.e. crack in a shell or loss of a limb), the granular amebocyte undergoes aggregation, adhesion, and degranulation in response to LPS. The granule contents are released into the hemolymph where they form an insoluble gel (Iwanaga et al., 1992).
Coagulogen is the coagulation factor in invertebrates released from the granular amebocytes that is proteolytically converted into an insoluble gel-like coagulin polymer through non-covalent self-polymerization (Kawasaki et al., 2000). Roth et al. (1989) demonstrated the non-covalent interaction in the coagulin polymers of Limulus polyphemus clots; the gelled lysates demonstrated rapid solubilization in monochloracetic acid, a property of clots that have not been covalently stabilized. Transformation of coagulogen into an insoluble coagulin was discovered and documented in the American horseshoe crab, Limulus polyphemus and characterized by Mosesson et al. (1979), and for the purposes of this review, the Limulus hemolymph coagulation mechanism will serve as a model for comparison of the coagulation systems of vertebrates and invertebrates. The coagulation mechanism in various horseshoe crab species is very similar, but the coagulogen factor of Limulus polyphemus is immunologically different from that of Tachypleus tridentatus and Tachypleus gigas (Shishikura and Sekiguchi, 1978).
Coagulin polymers are stabilized when plasma transglutaminase (TGase) and phenoloxidase intermolecularly cross-link the chains of coagulin. In crustaceans, hemolymph coagulation depends on the TGase-mediated cross-linking of the plasma clotting proteins, but, as opposed to vertebrates, this TGase activity is free of the proteolytic cascade (Osaki and Kawabta, 2004). TGases play important roles during the final stage of blood coagulation in both crustaceans (coagulin polymerization) (Omori et al., 1991) and mammals (fibrin polymerization) (Osaki et al., 2002), which suggests a relationship between fibrin and coagulin. Phenoloxidase is not found in vertebrates.
The proteolytic cascade in non-insect arthropods
The molecular cascade of invertebrate blood coagulation in non-insect arthropods (i.e. Limulus polyphemus) consists of four serine protease zymogens (Factor C, Factor G, Factor B, and Proclotting factor) and one clottable protein (coagulogen) (Iwanaga et al., 1992; Nakamura et al. 1982; and Tatsushi et al. 1995). Factors C and G exist on hemocyte surfaces, are sensitive to PAMP, and act as pattern recognition proteins for LPS and 1-3-(-D-glucans, respectively (Ariki et al., 2003 and Tatsushi et al. 1995). Factor C, when stimulated by LPS, activates Factor B and triggers exocytosis of other coagulation factor-containing granules in the hemocyte by a heterotrimeric GTP-binding protein-mediated signaling pathway that involves inositol triphosphate (IP3) leading to an increase in intracellular Mg2+ and Ca2+ (Ariki et al, 2003). Activated Factor B then converts Proclotting factor to Clotting factor, and Clotting factor converts soluble coagulogen to insoluble coagulin (Nakamura et al., 1986; Roth and Levin, 1992; and Fortes-Dias et al., 1993). The coagulogen monomers are then stabilized in polymer form by transglutaminases and phenoloxidase. When stimulated by 1-3-(-D-glucans, Factor G can activate Proclotting factor directly, converting it to Clotting factor (Tatsushi et al., 1995). The resulting agglutination at the end of the cascade ensnares the microbial pathogen, and even prevents Brownian motion in the trapped bacteria (Bang, 1956). (See Figure 2.)
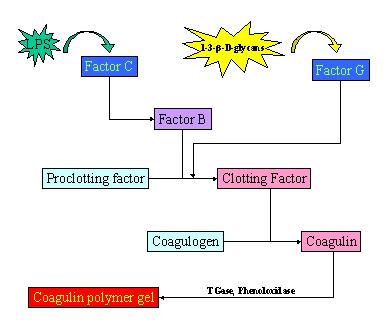
Figure 2. The proteolytic coagulation cascade in invertebrates. The invertebrate blood coagulation cascade is similar to the vertebrate cascade in that it is a series of proteolytic activations that lead to the production of a clot. The cascade is much simpler, though, consisting of only 8 proteolytically cleaved factors. Primitive arthropods such as horseshoe crabs utilize this method of hemolymph coagulation, but the exact pathway for a cascade of insect hemolymph coagulation is currently unknown.
Proteolytic cleavage in the Limulus and Limulus-like (chelicerate) cascades also releases products that act as antimicrobial agents (Iwanaga, 1998). Secreted from the granules of hemocytes, along with the coagulation factors, is prophenoloxidase, which is converted to its active form, phenoloxidase, by the peptidoglycan cell wall components of bacteria (Lee et al., 2004), which stimulate peptidoglycan recognition proteins (PGRPs) in the hemocyte (Dziarski, 2003). PGRPs act as both recognition molecules and signaling receptors that demonstrate specificity for various microbial infections. Phenoloxidase (monophenol- l-dopa oxygen oxidoreductase) is a multicopper oxidase, which, in addition to stabilizing coagulin polymers, plays an important role in melanin synthesis, necessary for defense against intruding microorganisms and parasites, wound healing and cuticle pigmentation (Zufelato et al., 2004). A cascade of reactions involving the tyrosine-phenyloxidase pathway causes the melanization of the capsule and the death of the invading particle (Fisher, 1963). The rate of phenyloxidase melanization activity was found to be regulated by glucose and nitrogen catabolite repression (Nurudeen and Ahearn, 1979). Lee et al. (2004) demonstrated that cleavage of crayfish hemocyanin subunit 2 at the N-terminal part results in that the processed hemocyanin exhibits phenoloxidase activity. Interestingly, cross-linking of the clot may be dependent on hemocyanin that assumes phenoloxidase activity through interaction with the clotting factors (Nagai and Kawabata, 2000).
Invertebrates are poikilotherms and lack a mechanism for self-control of body temperature hemostasis. Changes in body condition, to include temperature, may be responsible for variations in clotting response. Chen et al. (1993) observed that concentrations of coagulogen changed with respect to season, molting stages, and trauma conditions in shrimps. Since coagulation of the hemolymph is such an important physiological mechanism of hemostasis as well as immune defense, it is logical that some invertebrates may have evolved a mechanism for the regulation of this process. During exocytosis of the amebocyte granules in Limulus, coagulation inhibitors are released, including small quantities of three inhibitory chemicals: a human (2-macroglobulin homolog, a low molecular weight acid- and heat-stable inhibitor, and an acid-labile inhibitor. Of the three, only the acid-labile inhibitor is capable of coagulation inhibition (Armstrong et al., 1984). These findings suggest a regulatory control of the invertebrate coagulation system, and this idea is further reinforced by the recent discovery that environmental estrogens rapidly modulate hemocytes in mussels through activation of transduction pathways involving different kinase-mediated cascades (Canesi, et al., 2004).
Insect hemolymph coagulation
Many features of insect hemolymph coagulation have been and remain a mystery. Early observations include characterization of a fibrinogen-equivalent factor (later coined coagulogen) in the hemolymph of the locust, Locusta migratoria (Brehelin, 1979). By the 1980s, the fibrinogen-like coagulogen had been described in a number of insects. Characterization of these proteins and analysis of their control were performed in the cockroach, Rhyparobia-maderae blattaria (Ferstl et al., 1988, and Bohn and Saks, 1986).
Recent advances in genomic sequence technology allow for detailed analysis of presently known insect coagulation factors and facilitate the search for vertebrate-homologous domains within the genetic code of insects. Domains in clotting factors of insects have shown homology with vertebrate Factors V and VIII and von Willebrand Factor (vWF), as well as epidermal growth factor (EGF) and sushi domains (Goto et al., 2001, and Kotani et al., 1995). Fibrin-like genes were also found in the genomes of the fruit fly, Drosophila melanogaster, and the mosquito, Anopheles gambiae (Adams et al., 2000).
Drosophila and Anopheles genomic data pose some striking features that may lead to the elucidation of an insect hemolymph coagulation cascade. In Drosophila, the cascade involved in creating dorso-ventral polarity shares organizational similarities to the proteolytic, hemolymph coagulation cascade in vertebrates; both systems have a functional core consisting of three required serine proteases (referred to as the upstream, middle, and downstream proteases) Krem and Cera, 2002). The dorso-ventral cascade in Drosophila also bears relation to the hemolymph coagulation in horseshoe crabs (Krem and Cera, 2002), but examination of the proteases for homology is difficult because the Drosophila genome contains approximately 200 serine proteases or protease homologues (Ross et al., 2003). Though the mechanisms of hemolymph coagulation in Drosophila (and insects in general) is still largely unknown, the ability of Drosophila to heal and seal wounds has served as a model for the study of insect would closure (Jacinto et al., 2001, and Jacinto and Martin, 2001).
CONCLUSIONS
Researchers have long sought to elucidate a biochemical evolutionary trend that explains the appearance of the proteolytic coagulations cascades. In early observations of the vertebrate and invertebrate blood coagulation, the similarities of the of the cascades, especially with regard to the physiological natures of fibrinogen and coagulogen, was a compelling link between both classes of organisms. After decades of analysis, the relationship of organizational structure of the respective coagulation cascades appears to be only coincidental in that they each evolved by accumulation of substrates in a chain of proteolytically activated enzymes; however, since coagulation in both vertebrate and invertebrate systems is reliant on proteolytic cleavage, and intramembrane proteolysis is a control mechanism that has been evolutionally conserved across a lineage as vast as that of bacteria to humans, it is logical that this distant connection is a relevant evolutionary starting point for explaining both the conserved and divergent aspect of the proteolytic clotting cascades.
Moving on from bacterial prokaryotes to eukaryotic, multicellular organisms, the increasing complexity of biological systems is responsible for the need to develop defensive mechanisms against invaders (i.e. the simple prokaryotes). The fact that all multicellular organisms possess innate immunity to various pathogens suggests that the development of protective biological measures began shortly after the evolutionary divergence of single-celled to multicellular organisms. This fact may help to explain the conservation of the blood coagulation system among the largely diverse classes of vertebrates. As for the invertebrate coagulation systems, the mechanisms involved in clotting physiology seem as diverse as invertebrates themselves. The differences in hemolymph coagulation of primitive arthropods like horseshoe crabs and that of insects, as well as the high number of serine protease enzymes in Drosophila, suggest a significant event in the evolution of insects that required accumulation of genes for a complex, defensive physiology.
It is logical that if the innate defense is an evolutionary product of the divergence from single-celled to multicellular organisms, then there must be some correspondence in the evolution of the coagulation cascades. For vertebrates, Doolittle et al. (1997) proposed that the best candidates for spawning of prototypic fibrinogen are lectins, and based on this theory, Kairies et al. (2001) demonstrated the first evidence of a common ancestor for innate immunity and the blood coagulation systems by examining the crystal structure of tachylectin 5A (TL5A). TL5A, a non-self recognizing lectin from the hemolymph plasma of the horseshoe crab, T. tridentatus, contains not only a common fold but also related functional sites with the (-fragment of fibrinogen. This small site homology among proteins of vertebrates and invertebrates may be the link between the coagulation systems, and therefore, may usher a new generation of research to elucidate the origin of the homologous fragments.
Even with the newly discovered homology of coagulation protein fragments, it still seems more likely that the coagulation systems evolved separately and simply have small similarities in structure of the peptides. If the evolutionary relationship proposed by Kairies et al. (2002) were significant based on Doolittle et al.'s (1997) proposal about lectins, then the appearance of any lectin in the plasma of an invertebrate would be an evolutionary marker relating the innate immunity and blood coagulation. With respect to the major proteins in the cascade of invertebrate blood coagulation, namely coagulogen, there is a significant homology with nerve growth factor (NGF) (Bergner et al., 1996), which further emphasizes that this neutrophin fold-containing protein and its insect homologues evolved separately from the vertebrate proteolytic cascades. Further study in the relationship between the vertebrate and invertebrate coagulation systems will follow this review.
ACKNOWLEDGEMENTS
I would like to thank Dr. John Doucet and Dr. Enmin Zou, both of Nicholls State University in Thibodaux, LA, for their guidance in the creation of this review. Special thanks are also in order for Dr. Ulrich Theopold of the University of Stockholm, Sweden, for his generosity in sharing scientific data. This review is dedicated to my parents, David and Elaine Bonvillain, for their unconditional love and support.
REFERENCES
Adams, M.D et al. 2000. The genome sequence of Drosophila melanogaster. Science. 287: 2185-2195.
Alberts, B.; Johnson, A.; Lewis, J.; Raff, M.; Roberts, K.; and Walter, P. Molecular Biology of the Cell. 4th ed. pp. 1284. New York. Garland Science. 2002.
Antonarakis, S. E.; Copeland, K. L.; Carpenter, R. J., Jr.; Carta, C. A.; Hoyer, L. W.; Caskey, C. T.; Toole, J. J.; Kazazian, H. H., Jr. 1985. Prenatal diagnosis of haemophilia A by factor VIII gene analysis. Lancet I: 1407-1409.
Ariki, S.; Koori, K.; Osaki, T.; Motoyama, K.; Inamori, K.; and Kawabata, S.; 2003. A serine protease zymogen functions as a pattern recognition receptor for lipopolysaccharides. PNAS. 101 (4): 953-958.
Armstrong, P.B.; Levin, J.; and Quigley, J.P. 1984. Role of endogenous proteinase inhibitors in the regulation of the blood clotting system of the horseshoe crab, Limulus polyphemus. Thromb. And Haemost. 52 (2): 117-120.
Bang, F. 1956. A Bacterial Disease of Limulus polyphemus. Reprinted with permission from Johns Hopkins Medical School. 2000.
Bardoni, B.; Sampietro, M.; Romano, M.; Crapanzano, M.; Mannucci, P. M.; Camerino, G. 1988. Characterization of a partial deletion of the factor VIII gene in a haemophiliac with inhibitor. Hum. Genet. 79: 86-88.
Berg, J.M., Tymoczko, J., and Stryer, L. Biochemistry. 5th ed. pp. 280. New York. W.H. Freeman and Company. 2002.
Bizzozero, J. 1882. Uber einen neuen formbestandtheil des blutes und dessen rolle bei der thrombose und der blutgerinnung. [A New Component of the blood and its role in blood clotting with the thrombose]. Virchows Arc. Pathol. Anat. Physiol. Klin. Med. 90: 261-332.
Briet, E.; Bertina, R. M.; van Tilburg, N. H.; Veltkamp, J. J. 1982. Hemophilia B Leyden: a sex-linked hereditary disorder that improves after puberty. New Eng. J. Med. 306: 788-790.
Brocker-Vriends, A. H. J. T.; Briet, E.; Dreesen, J. C. F. M.; Bakker, B.; Reitsma, P.; Pannekoek, H.; van de Kamp, J. J. P.; Pearson, P. L. 1990. Somatic origin of inherited haemophilia A. Hum. Genet. 85: 288-292.
Brown, M.S.; Ye, J.; Rawson, R.B.; and Goldstein, J.L. 2000. Regulated intramembrane proteolysis: a control mechanism conserved from bacteria to humans. Cell. 100: 191-198.
Canesi, L.; Lorusso, L.; Ciacci, C.; Betti, M.; Zampini, M.; and Gallo, G. 2004. Environmental estrogens can affect the function of mussel hemocytes through rapid modulation of kinase pathways. Gen. and Comp. Endo. 138 (1): 58-69.
Chen, Y.; Huang, S.; Cheng, J.; and Tsai, I. 1993. Relationship between hemolymph coagulation and disease in shrimps: Purification and characterization of the hemolymph coagulogen of Penaeids. Rep. Fish Disease Res. 0 (13): 1-9.
Coursey, Y; Ahmad, N.; McGee, B.M.; Steimel, N.; and Kimble, M. 2003. Amebocyte production begins at stage 18 during embryogenesis in Limulus polyphemus, the American horseshoe crab. Biolog. Bulletin. 204 (1): 21-27.
Davie, E. and Ratnoff, O. 1964. Waterfall sequence for intrinsic blood clotting. Science. 145: 1310-1312.
Doolittle, R.F., Spraggon, G., and Everse, S.J. 1997. Evolution of vertebrate fibrin formation and the process of its dissolution. Ciba. Found. Symp. 212: 4-17.
Dziarski, R. 2003. Recognition of bacterial peptidoglycan by the innate immune system. Cell. Mol. Life Sci. 60: 1793-1804.
Esmon. C.T. 2000. Regulation of blood coagulation. Biochem. Biophys. Acta. 1477: 349-360.
Fisher, R.C. 1963. Oxygen requirements and the physiological suppression of supernumary insect parasitoids. J. Exp. Biol 38: 605-658.
Fortes_Dias, C.; Minetti, C.; Lin, Y.; and Liu, T. 1993. Agglutination activity of Limulus polyphemus coagulogen following limited proteolysis. Comp. Biochem. And Physiol. B Comp. Biochem. 105 (1): 79-85.
Giannelli, F.; Green, P. M.; High, K. A.; Sommer, S.; Lillicrap, D. P.; Ludwig, M.; Olek, K.; Reitsma, P. H.; Goossens, M.; Yoshioka, A.; Brownlee, G. G. Haemophilia B: database of point mutations and short additions and deletions--third edition, 1992. Nucleic Acids Res. 20: 2027-2063; 1992.
Girolami, A.; Molaro, G.; Lazzarin, M.; Scarpa, R.; Brunetti, A. 1970. Congenital haemorrhagic condition similar but not identical to factor X deficiency: a haemorrhagic state due to an abnormal factor X? Scand. J. Haemat. 7: 91-99.
Goto, A. et al. 2001. A Drosophila haemocyte-specific protein, hemolectin, similar to human von Willebrand factor. Biochem. J. 359: 99-108.
Hadley, M.E. Endocrinology. 5th ed. pp. 75. Upper Saddle River. Prentice-Hall. 2000.
Hayem, G. On the mechanism of blood clotting. 1882. C.R. Acad. Sci. 95: 18-21.
Higuchi, M.; Kochhan, L.; Olek, K. 1988. A somatic mosaic for haemophilia A detected at the DNA level. Molec. Biol. Med. 5: 23-27.
Hurtubise, P. E.; Coots, M. C.; Jacob, D. J.; Muhleman, A. F.; Glueck, I.1979. A monoclonal IgG(4)-gamma with factor V inhibitory activity. Journ. Immun. 122: 2119-2221.
Iwanaga, S. et al. 1998. New types of clotting factors and defense molecules found in horseshoe crab hemolymph: their structures and functions. J. Biochem. (Tokyo) 123: 1-15.
Iwanaga, S.; Miyata, T.; Tokunaga, F.; and Muta, T. 1992. Molecular mechanism of hemolymph clotting system in Limulus. Thrombosis Research. 68 (1): 1-32.
Jacinto, A. and Martin, P. 2001. Morphogenesis: unraveling the cell biology of hole closure. Curr. Biol. 11: R705-R707.
Jacinto, A. et al. 2001. Mechanisms of epithelial fusion and repair. Nat. Cell Biol. 3: E117-E123.
Jacobs DS et al, ed. The Laboratory Test Handbook, 5th ed.. pp. 327-358. Cleveland, Lexi-Comp. 2001.
Jiang, Y. and Doolittle, R. 2003. The evolution of vertebrate blood coagulation as viewed from a comparison of puffer fish and sea squirt genomes. Proc. Natl. Acad. Sci. USA. 100(13): 7527-532.
Kairies, N.; Biesel, H.; Fuentes-Prior, P.; Tsuda, R.; Muta, T.; Iwanaga, S.; Bode, W.; Huber, R.; and Kawabata, S. 2001. The 2.0 Ã… crystal structure of tachylectin 5A provides evidence for the common origin of the innate immunity and the blood coagulation systems. Proc Natl Acad Sci USA. 98(24): 13519-13524.
Kawasaki, H.; Nose, T.; Muta, T.; Iwanaga, S.; Shimohigashi, Y; and Kawabata, S. 2000. Head-to-tail polymerization of coagulin, a clottable protein of the horseshoe crab. J. Bio. Chem. 275 (45): 35297-35301.
Kazazian, H. H., Jr.; Wong, C.; Youssoufian, H.; Scott, A. F.; Phillips, D. G.; Antonarakis, S. E. 1988. Haemophilia A resulting from de novo insertion of L1 sequences represents a novel mechanism for mutation in man. Nature 332: 164-166.
Kerr, R.; Stirling, D.; and Ludlam, C.A. 2001. Interleukin-6 and haemostasis. Br. J. Haematol. 115: 3-12.
Ketterling R.P.; Vielhaber E.; Li, X.; Drost J.; Schaid D.J.; Kasper C.K.; Phillips J.A. 3rd; Koerper M.A.; Kim H.; Sexauer C.; Gruppo R.; Ambriz R.; Paredes R.; Sommer S.S. 1999. Germline origins in the human F9 gene: frequent G:C-->A:T mosaicism and increased mutations with advanced maternal age. Hum Genet 105:629-40
Klung, W.S., and Cummings, M.R. Essentials of Genetics. 4th ed. pp.269. Upper Saddle River. Prentice-Hall, Inc. 2002.
Kotani, E. et al. 1995. Cloning and expression of the gene of hemocytin, an insect humoral lectin which is homologous with the mammalian von Willebrand factor. Biochem. Biophys. Acta. 1260: 245-258.
Krem, M.M and Cera, E.D. 2002. Evolution of enzyme cascades from embryonic development to blood coagulation. Trends Biochem. Sci. 27: 67-74.
Lee S.Y.; Lee B.L.; and Soderhall K. 2004. Processing of crayfish hemocyanin subunits into phenoloxidase. Biochem. Biophys. Res. Commun. 322 (2): 490-496.
Lee, M.; Osaki, T.; Lee, J.; Baek, M.; Zhang, R.; Park, J.; and Kawabata, S. 2004. Peptidoglycan recognition proteins involved in 1,3-beta-d-glycan-dependent prophenoloxidase activation system of insect. J. Biol. Chem. 279 (5): 3218-3227.
Liu, T.; Chen, Y.; and Chiou, S. 1999. Human and Limulus Blood: Opportunity for Development of a Medical Biotechnology Project in Taiwan. J. Chin. Chem. Soc. 46 (3): 263-270.
Macfarlane, R. 1964. An enzyme cascade in the blood clotting mechanism and its function as a biochemical amplifier. Nature. 202: 498-499.
Mosesson, M.W.; Wolfenstein-Todel, C.; Levin, J.; and Bertrand, O. 1979. Characterization of amebocyte coagulogen from the horseshoe crab, Limulus polyphemus. Thrombosis Research. 14 (4-5): 765-780.
Muta T.; Seki N.; Takaki, Y.; Hashimoto, R.; Oda, T.; Iwanaga, A.; Tokunaga, F. ; and Iwanaga, S. 1995. Purified Horseshoe Crab Factor G: reconstitution and characterization of the 1-3-beta-D-glucan-sensitive serine protease cascade. Am. Soc. Biochem. Mol. Biol. Inc. 270 (2): 892-897.
Nagai, T. and Kawabata, S. 2000. A link between blood coagulation and prophenoloxidase activation in arthropod host defense. J. Biol. Che. 275: 29264-29267.
Nakamura, S. and Levin, J. 1982. Fractionation of Limulus amebocyte lysate characterization of activation of the pro-clotting enzyme by an endotoxin mediated activator. Biochem. Et Biophysica Acta. 707 (2): 217-225.
Nakamura, S.; Morita, T.; Harada-Suzuki, T.; Iwanaga, S.; Takahashi, K.; and Niwa, M. 1982. A clotting enzyme associated with the hemolymph coagulation system of horseshoe crab Tachypleus tridentatus: its purification and characterization. J. Biochem. (Tokyo). 92 (3): 781-792.
Nakamura, T.; Horiuchi, T.; Morita, T.; and Iwanaga, S. 1986. Purification and properties of intracellular clotting factor, Factor B, from horseshoe crab, Tachypleus tridentatus hemocytes. J. Biochem. (Tokyo). 99 (3): 847-858.
Nurudeen, T.A. and Ahearn, D.G. 1979. Regulation of melanin production by Cryptococcus neoformans. J. Clin. Microbiol. 10 (5): 724-729.
Omori, S.; Chong, C.; Hoodbhoy, T.; and Mckrell, N. 1991. Localization and roles of coagulogen and transgultaminase in hemolymph coagulation in decapod crustaceans. Comp. Biochem. And Physiol. B. 100 (3): 517-522.
Osaki, T. and Kawabata, S. 2004. Structure and function of coagulogen, a clottable protein in horseshoe crabs. Cell. Mol. Life Sci. 61 (11): 1257-1265.
Osaki, T.; Okino, N.; Tokunaga, F.; Iwanaga, S.; and Kawabata, S. 2002. Proline-rich cell surface antigens of horseshoe crab hemocytes are substrates for protein cross-linking with a clotting protein, coagulin. J. Biol. Chem. 277 (42): 40084-40090.
Randall, D., Burggren, W., and French, K. Animal Physiology: Mechanisms and Adaptations. 5th ed. pp. 475, 522, 532. New York. W.H. Freeman and Company. 2002.
Raven, P.H. and Johnson, G.B. Biology. 5th ed. pp. 958, 986. Boston. McGraw-Hill. 1999.
Reitsma, P. H.; Bertina, R. M.; Ploos van Amstel, J. K.; Riemens, A.; Briet, E. 1988. The putative factor IX gene promoter in hemophilia B Leyden. Blood 72: 1074.
Roberts, H.R.; Monroe, D.M.; Oliver, J.A.; Chang, J.Y.; and Hoffman, M. Newer concepts of blood coagulation. 1998. Haemophilia. 4: 331-334.
Ross, J. et al. 2003. Serine proteases and their homologues in the Drosophila melanogaster genome: an initial analysis of sequence conservation and phylogenetic relationships. Gene. 304: 117-131.
Roth, R. and Levin, J. 1992. Purification of Limulus polyphemus proclotting enzyme. J. Biol. Chem. 267 (33): 24097-24102.
Roth, R.I.; Chen, J.C-R.; and Levin, J. 1989. Stability of gels formed following coagulation of Limulus amebocyte lysate lack of covalent cross-linking for coagulin. Thrombosis Research. 55 (1): 25-36.
Shigenaga, T.; Takayenoki, Y.; Kawasaki, S.; Seki, N.; and Iwanaga, S. 1993. Separation of large and small granules from horseshoe crab (Tachypleus tridentatus) hemocytes and characterization of their components. J. Biochem. (Tokyo). 114 (3): 307-316.
Shishikura, F. and Sekiguchi, K. 1978. Comparative study on horseshoe crab coagulogens. J. Exp. Zool. 206 (2): 241-246.
Taylor, S. A. M.; Duffin, J.; Cameron, C.; Teitel, J.; Garvey, B.; Lillicrap, D. P. 1992. Characterization of the original Christmas disease mutation (cysteine 206serine): from clinical recognition to molecular pathogenesis. Thromb. Haemost. 67: 63-65.
ten Cate, H.; Biemond, B.J.; Levi, M.; Wuillemin, W.A.; Bauer, K.A.; Barzegar, S.; Buller, H.R.; Hack, C.E.; ten Cate, J.W.; and Rosenberg, R.D. 1996. Factor Xia induced activation of the intrinsic cascade in vivo. Thromb. Haemost. 75: 445-449.
Theopold, U.; Schmidt, O.; Soderhall, K.; and Dushay, M.S. 2004. Coagulation in arthropods: defense, wound closure, and healing. Trends in Immunology. 25 (6): 289-294.
Thompson A.R.; Chen S.H. 1993. Characterization of factor IX defects in hemophilia B patients. Methods Enzymol. 222:143-69.
Veltkamp, J. J.; Meilof, J.; Remmelts, H. G.; Van der Vlerk, D.; Loeliger, E. A. 1970. Another genetic variant of haemophilia B: haemophilia B Leyden. Scand. J. Haemat. 7: 82-90.
Versteeg, H.H.; Peppelenbosch, M.P.; and Spek, C.A. 2001. The pleiotropic effects of tissue factor: a possible role for factor VIIa-induced intracellular signaling? Thromb. Haemost. 86: 1353-1359.
von dem Borne, P.A.; Meijers, J.C.; and Bouma, B.N. 1995. Feedback activation of factor XI by thrombin in plasma results in additional formation of thrombin that protects fibrin clots from fibrinolysis. Blood. 86: 3035-3042.
von Willebrand, E. A. 1931. Ueber hereditaere Pseudohaemophilie. Acta Med. Scand. 76: 521-550.
Weinmann A.F.; Murphy M.E.; Thompson A.R. 1998. Consequences of factor IX mutations in 26 families with haemophilia. B. Br. J. Haematol. 100: 58-61.
Windsor, S.; Lyng, A.; Taylor, S. A. M.; Ewenstein, B. M.; Neufeld, E. J.; Lillicrap, D. 1995. Severe haemophilia A in a female resulting from two de novo factor VIII mutations. Brit. J. Haemat. 90: 906-909.
Woods-Samuels, P.; Wong, C.; Mathias, S. L.; Scott, A. F.; Kazazian, H. H., Jr.; Antonarakis, S. E. 1989. Characterization of a nondeleterious L1 insertion in an intron of the human factor VIII gene and further evidence of open reading frames in functional L1 elements. Genomics 4: 290-296.
Youssoufian, H.; Antonarakis, S. E.; Bell, W.; Griffin, A. M.; Kazazian, H. H., Jr. 1988. Nonsense and missense mutations in hemophilia A: estimate of the relative mutation rate at CG dinucleotides. Am. J. Hum. Genet. 42: 718-725.
Youssoufian, H.; Kazazian, H. H., Jr.; Phillips, D. G.; Aronis, S.; Tsiftis, G.; Brown, V. A.; Antonarakis, S. E. 1986. Recurrent mutations in haemophilia A give evidence for CpG mutation hotspots. Nature 324: 380-382.
Zufelato, M.S.; Lourenco A.P.; Simoes Z.L.; Jorge J.A.; and Bitondi M.M. 2004. Phenoloxidase activity in Apis mellifera honey bee pupae, and ecdysteroid-dependent expression of the prophenoloxidase mRNA. Insect Biochem. Mol. Biol. 34 (12): 1257-1268.
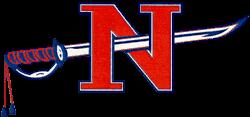