(last updated: Feb 24, 2009)
The Global Carbon Cycle - Where is the Carbon?
The global carbon cycle is examined in order to compare human inputs
with the natural background. The flows described are effective on
the timescale of one year.
Making sense of
measurement units:
- When describing the carbon cycle, only the weight of
the carbon is measured. The rest of the compound (such as the
oxygen part of carbon dioxide) is only "going along for the ride", so
it is
ignored. We follow the carbon as it moves through its cycle
of different compounds.
- Quantities are measured in gigatonnes. One
Gigatonne (Gt) = One Petagram (Pg) = 1015
grams
= 1 cubic kilometer of water.
- Carbon dioxide levels in the atmosphere are often
measured in parts per million (ppm). One ppm of CO2
in the air is
equivalent to 2.12 Gt of carbon.
- Carbon
dioxide is also measured as a partial pressure of the atmosphere.
Today's level of 380 ppm is equivalent to 380 µAtm (micro atmospheres)
or 0.38 millibars.
- Given that CO2 has
risen by 100 ppm, that means we have added about half a kilogram of
carbon to the atmosphere over every square meter.
- Each barrel of oil releases 120 kg of
carbon. 8.5 billion barrels of oil contain one Gt of
carbon. 112 Gt of oil has been extracted so far.
|
The picture below illustrates the flow of carbon compounds between
various components of the ecosphere.
The
arrows show the annual flow, in Gigatones per year, while the boxes
indicate "sinks" where carbon is stored. For example, reading from the
top right of the chart, 6.2
Gigatonnes per year of carbon (as part of carbon dioxide) from fossil
fuels flow into the
atmosphere, which contains 775 Gigatonnes of carbon.
This
chart is rather out of date, today nearly 8 Gt of carbon is emitted,
into an atmospheric sink of 830 Gt.
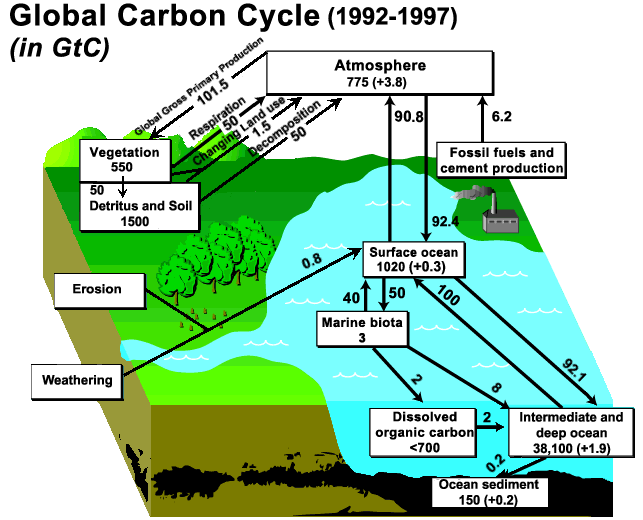
The
geological carbon cycle
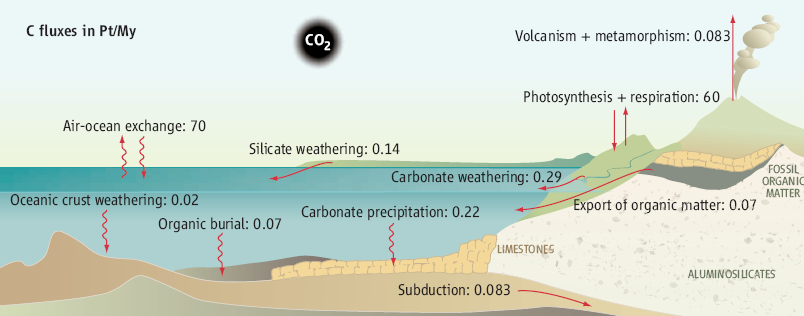
The natural regulation of
atmospheric CO2
implies different carbon reservoirs playing roles on different time
scales. Ocean and biomass reservoirs play a role at time scales lower
than a couple of thousand years. At longer (geological) time scales,
carbonate weathering is balanced by carbonate precipitation in the
ocean, but the volcanic input of CO2 to the
atmosphere is only compensated by the weathering of Ca-Mg silicate
minerals in soils (CaSiO3 + CO2
= CaCO3 + SiO2).
For each mol of C precipitated into carbonate, a mol of C is released
to the atmosphere, and the net sequestration of atmospheric carbon is
0.07 PgC/year. The organic sequestration in the form of fossil organic
matter buried in sediments is thought to be compensated by the
oxidation of ancient organic matter on land (not shown). New findings (4,
5)
in the Himalaya lead to a new flux of CO2
degassing in mountain ranges and make mountains a locus of CO2
production instead of CO2 consumption. Fluxes in
PgC/year or PtC/My. [Science
July 2008]
Other human sources of carbon dioxide (not shown in the chart)
- Human breathing (at 1kg/day per person) is 0.6 Gt
per year. This counts as part of the 50 Gt respiration part of the
cycle, and should not be thought of as 10% of fossil fuel emissions.
- Volcanic activity now
releases about 0.13 to 0.23
Gt of carbon dioxide each year, which is about 1% of the
amount which is released by human activities. The natural
carbon
dioxide level is maintained by volcanic activity over a time scale of
millions of years, but has little effect on the scale of a century.
Human activity emits around 8.5 Gigatonnes of carbon per year (or 3.3
ppm) into
the
atmosphere (in 2006),
which is about 3% of the natural exchange involving the ocean and land
vegetation. The observed
increase of CO2
in the atmosphere from about
280 ppm in the preindustrial era to 315 ppm when accurate
measurement began in 1958, to 378 ppm in 2004 (see How do we
know), now averaging about 1.9 ppm
(or 3.3 Gt) / year, or 212 Gt total. About 45% of
humanity’s carbon production has remained
in
the
atmosphere, with a less certain division between the terrestrial
biosphere and oceans (2.0 ± 0.8 Gt).
The ratio of the annual CO2
increase in the atmosphere to the annual CO2
emissions, the airborne fraction, varies a lot from year to year, but
it has averaged about 58% for half a century with no obvious trend. The
other 42% must be taken up by the ocean, the vegetation, and the soils.
The ocean is thought to take up about 20-35%, leaving 5-20% as the net
sink in vegetation and soil. Vegetation and soils are also a source
of CO2
via deforestation and biomass burning, so this refers to their net
effect.
[Hansen
2005]
Other facts about the carbon cycle:
- For 1990 to 1999, the ocean-atmosphere flux is estimated as
-1.7
± 0.5 GtC/yr and the land-atmosphere flux as -1.4
± 0.7 GtC/yr. [IPCC
2001]
- The net CO2 release due to land-use
change
during the 1980s has been estimated as 0.6 to 2.5 GtC/yr (central
estimate 1.7 GtC/yr). This net CO2 release is
mainly due to deforestation in the tropics. Uncertainties about
land-use changes limit the accuracy of these estimates. [IPCC
2001]
- Carbon is removed from the cycle by both biological and
geological
processes. The biological rate is 200 times the
geological
rate.
- Photosynthetic marine organisms fix about 50 Gt of carbon
per year into
their bodies. Benthic (bottom-dwelling) photosynthetic organisms, such
as seaweed, sea grasses and corals, fix about 1 Gt of carbon
per
year.
- Total carbon stored in northern peatlands has been
estimated as about
455 GtC [ref]
- About twice as much terrestrial
carbon is received by inland waters as reaches the world's oceans. In
total, inland waters may bury about four times as much carbon as do the
oceans. [Science Feb 2009]
Forests
Forests cover 42 million km2
in tropical, temperate, and boreal lands, about
30% of the world's land surface. Forests influence
climate through exchanges of energy, water, carbon dioxide,
and other chemical species with the atmosphere. Forests
store 45% of terrestrial carbon. Carbon uptake by forests
contributed to a "residual" 2.6 GtC/yr terrestrial carbon
sink in the 1990s, about one third of anthropogenic carbon emission
from fossil fuel and land-use change. [Science
June 2008] The average absorption of a U.S.
commercial forest is 0.8
tons of carbon/hectare/year [ref].
Boreal forest fires add to warming initially, as greenhouse
gases
are released, but the increased exposure of snow in burned areas
produces a delayed reflection that induces cooling. This
result implies that future increases in boreal fire may
not accelerate climate warming. [Science
Nov 2006].
But because global warming will reduce the area covered by snow, forest
fires may have more effect in the future.
Global forest fires burn an estimated 3 to 4.5 million km2
per year - about 4% of the vegetated land surface - and emit 2 to 3 Gt
of carbon into the atmosphere annually, equivalent to 30% of fossil
fuel emissions. [Science Aug 2008]
Coal Seam Fires
Wild coal fires are a global catastrophe
burning hundreds of millions of tonnes of coal every year and
contributing to climate change and damaging human health. These
fires can rage both above and below ground and may contribute more than
three per cent of the world's annual carbon dioxide emissions, which
are thought to be causing global warming. [ref]
Coal fires occur wherever there is coal, but major fires blaze in
Indonesia, China, India and the US. Alfred Whitehouse, of the Office of
Surface Mining in Jakarta, Indonesia, says there may be up to 1000
fires blazing underground in that country alone. 63 fires are
currently being monitored in the US. [ref]
Dr Glenn Stracher, of East Georgia College, Swainsboro, Georgia, said
coal field fires accounted for an estimated one to two per cent of
world emission of carbon dioxide from burning fossil fuels. "This is
equivalent to the carbon dioxide emitted each year from all the
vehicles in the United States," he said. [ref]
Andries Rosema of the Environmental Analysis and Remote Sensing (EARS)
firm in the Netherlands worked with colleagues using satellite and
airplane data to analyze the damage uncontrolled fires caused in China.
The team found that the fires released up to 360 million metric tons of
carbon dioxide — 2 to 3 percent of worldwide production per
year from burning fossil fuels, an amount equivalent to that emitted
per year from all automobiles and light trucks in the United States. [ref]
Carbon
Dioxide and Acid Rain
When water is exposed to
the air, substances in the air dissolve in the
water. Carbon dioxide is always present in air. It
dissolves by a series of reactions to form carbonic acid , H2CO3
(the acid that makes soda fizzy). The acid which is naturally
present in rain is carbonic acid.
CO2(g) + H2O(l)
<===> H2CO3(aq)
+ H2O<===> HCO3-(aq)
+ H3O+
Carbonic
acid is a weak acid, and produces few hydrogen ions in water.
Only about 0.3% of this acid is dissociated, so it is about 10 times
weaker than acetic acid. Therefore, even large concentrations
of
carbonic acid would not produce rain of very low pH. With the
current levels of carbon dioxide in the atmosphere (about 360 ppm in
1997), water naturally has a pH of about 5.6. This slight acidity is a
natural part of the transport of carbon in the environment.
Based
on ice core data, we know that the concentration of carbon dioxide in
the atmosphere has varied from a low of 280 ppm to the present high of
360 ppm over the past 100,000 years. During this time, the
natural pH of rain has remained between 5.7 and 5.6. Therefore rising
carbon dioxide levels in the atmosphere will have only a small effect
on the pH of rain. [ref]
Other illustrations of the carbon cycle:
The Distribution of Carbon on the Earth
The table below shows the relative amounts of each form of carbon on
the Earth. Observe that the biosphere contains about 1% of
the
world's organic carbon, most of which is fossil fuels or dissolved in
the ocean. The total amount of carbon dioxide in the ocean is
about 50 times greater than
the amount in the atmosphere, and is exchanged with the atmosphere on a
time-scale of several hundred years.
Carbon
Distribution on Earth
(All
values in Gigatonnes) |
All
Carbon on
Earth |
Organic
Carbon |
The
Biosphere |
|
Organic
Carbon
6.4 x 106 |
Non-organic
Carbonates
29.2 x 106 |
|
Biosphere:
5.5 x 104 |
Metamorphised
Sediment
1.4 x 106 |
Sediment
5 x 106 |
|
CO2
in the Air: 830 |
Plant
and
Animal Life: 610 |
Permafrost:
900
Soil
Organics, Peat: 1,500 |
Fossil
Fuel Deposits: 5,000
Shales: 7,000 |
Methane
Hydrates
500 - 3000 |
Ocean
Surface Layer: 600 |
Intermediate
Ocean: 7,000 |
Deep
Ocean
-
dissolved CO2
and carbonates
30,000 |
|
In the 1990s it was assumed that carbon quantities on the order of
10,000Gt C were stored in the form of methane hydrates (that equates to
around twice the entire fossil energy resource: Rogner, 1997), but
current estimates
suggest a much lower value (500–3000Gt C: Buffett and Archer,
2004; Milkov, 2004). [ref]
The amount of buried reduced carbon exceeds the amount of O2
in the
atmosphere by about a factor of 10. [Archer, ch 7]
Global Energy Balance
It is a remarkable fact that, averaged over the planet, the surface
receives more radiation from the atmosphere than directly from the sun!
To balance this extra input of radiation—the radiation
emitted by
atmospheric greenhouse gases and clouds—the earth’s
surface
must warm up and thereby emit more radiation itself. This is the
essence of the greenhouse
effect.
If air were not in motion, the observed concentration of greenhouse
gases and clouds would succeed in raising the average temperature of
the earth’s surface to around 85°F, much warmer than
observed. In reality, hot air from near the surface rises upward and is
continually replaced by cold air moving down from aloft; these
convection currents lower the surface temperature to an average of
60°F while warming the upper reaches of the atmosphere. So the
emission of radiation by greenhouse gases keeps the earth’s
surface warmer than it would otherwise be; at the same time, the
movement of air dampens the warming effect and keeps the surface
temperature bearable.
Below is the standard chart from the Intergovernmental Panel
on Climate Change (IPCC) of how energy from the sun is
absorbed by the Earth and/or radiated back into space.
Energy flow is measured in watts per square meter (W/m2
or Wm-2).
An increase of 1 W/m2
over the course of a year is the equivalent to an increase in the
energy content of the ocean of 2x1022
Joules. Regionally, ocean temperatures can vary by the
equivalent of 50 W/m2
over the course of two years. [ref]
It would take 8.5x1023
Joules to raise average temperature of the upper layer of the ocean
(down to 700 meters) by one degree Celsius.
Satellite measurements show that 235 W/m2
of incoming solar
radiation is absorbed by the Earth, but the latest models and
measurements suggest that the atmosphere is responsible for just
67 W/m2
of this amount. The rest is absorbed by the ground
and by
the oceans, which play a key role in the energy budget due to their
large heat capacity and their ability to store carbon dioxide, and, of
course, water vapour. The greenhouse effect is precisely the
difference between the long-wave radiation that is emitted by the
Earth’s surface and the upward thermal radiation that leaves
the
tropopause – the upper boundary of the turbulent portion of
the
atmosphere that we all inhabit. The greenhouse effect is about
146 W/m2
in clear skies and some 30 W/m2
higher
under cloud
cover. [ref]
The sun bathes the Earth in energy, continuously at a rate of about
173,000 terawatts. Photosynthesis captures about 100 TW per year.
Mankind is consuming about 13 TW of energy per year.
Earth's Internal Heat
83% of present surface heat flow is due to decay of radioactive
isotopes;
17% due to cooling of the Earth fom its initial molten
state. The mantle is cooling at
36°C/Ga;
3 billion years ago it was 150° C
hotter than present. Average heat loss through the surface is
87 milli-watts per square
meter (mW/m2),
with 101 mW/m2
in the ocean, and 65 mW/m2
under continents);
total 44x1012
W (from here).
Therefore at about 0.1 W/m2
the
Earth's internal heat is an insignificant part of the global energy
balance.
The Human Contribution to Climate Change
The chart below, from the IPCC
2007 Summary for Policy Makers,
shows the warming or cooling effect (radiative forcing) of greenhouse
gases and other influences on climate at the present time. Obseve
that most of the uncertainty comes from the cooling effects of aerosols
and clouds.
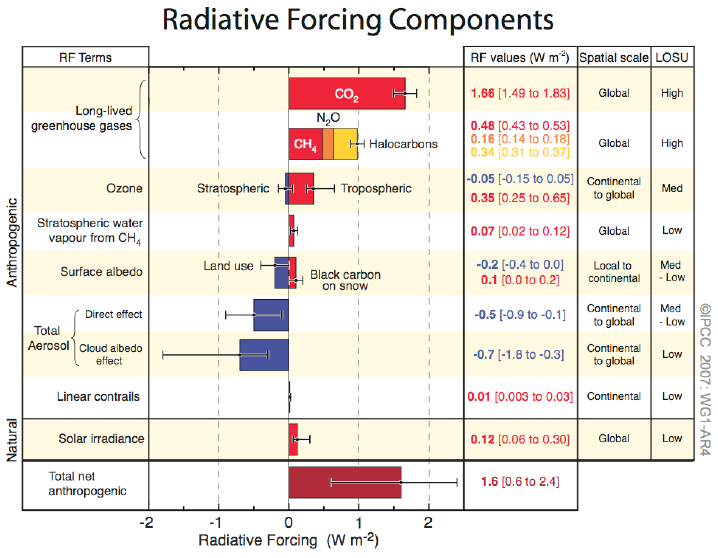
[Figure SPM-2] Global-average radiative forcing (RF)
estimates and ranges in 2005 for anthropogenic carbon dioxide (CO2),
methane (CH4), nitrous oxide (N2O)
and other important agents and mechanisms, together with the typical
geographical extent (spatial scale) of the forcing and the assessed
level of scientific understanding (LOSU). The net anthropogenic
radiative forcing and its range are also shown. These require summing
asymmetric uncertainty estimates from the component terms, and cannot
be obtained by simple addition. Additional forcing factors not included
here are considered to have a very low LOSU. Volcanic aerosols
contribute an additional natural forcing but are not included in this
figure due to their episodic nature. Range for linear contrails does
not include other possible effects of aviation on cloudiness.
These figures use an "abundance based" approach to measuring forcings,
meaning they are based on what is acually in the atmosphere now.
An alternative approach is called "emissions based", from Shindell
et al.
They point out that part of the methane emissions get
converted
into tropospheric ozone and carbon dioxide, and the real impact of
adding methane is nearly twice the IPCC value (0.79 W/m2 compared
to
0.48 W/m2).
Also, emissions of NOx have had an overall negative forcing,
as
the induced reduction in methane outweighs the increased ozone.
Therefore one should not use the values in the above table
alone
to determine which emisisons we should target to reduce global warming.
Human Sources of Greenhouse Gas
Emissions
This chart shows how human activity gets
coverted into
greenhouse gas emissions. It can be seen there is no single end use
that is responsible from most of the emissions. Reductions will need to
take place in all sectors.
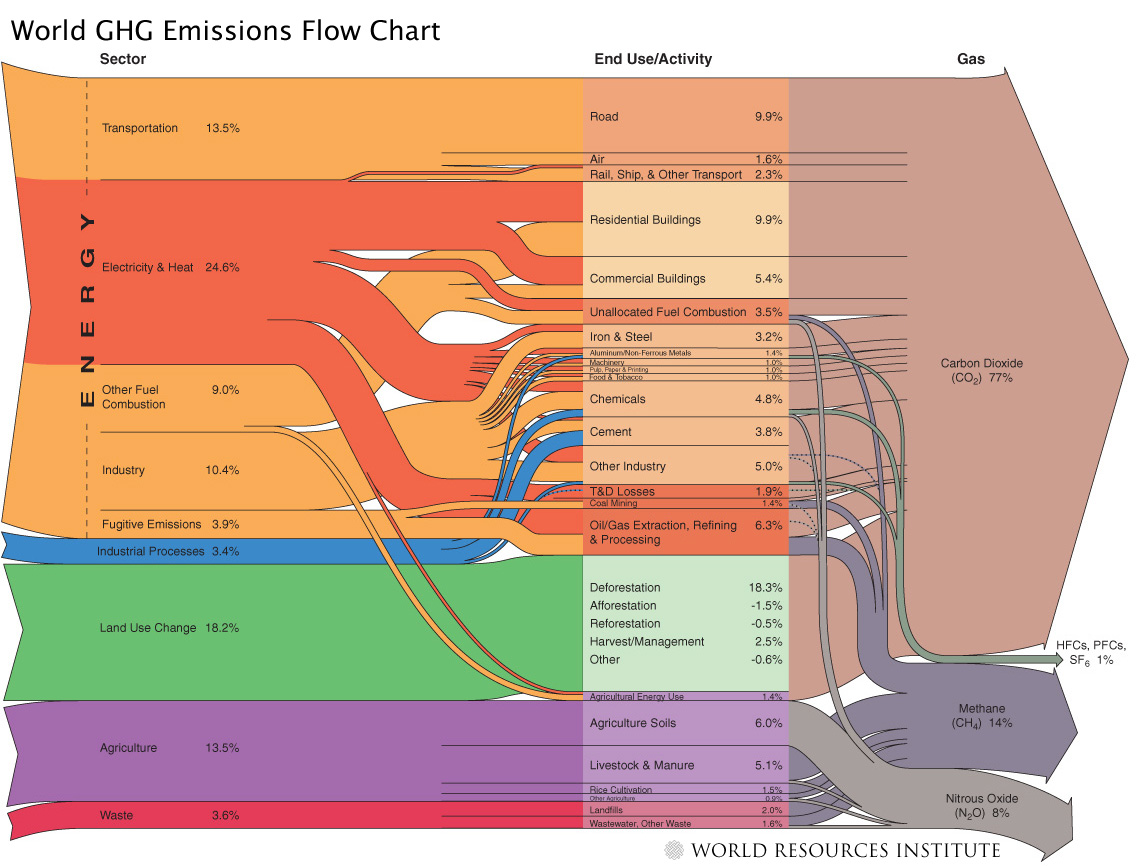
Climate Sensitivity and Feedbacks
Climate Sensitivity is the expected change in temperature caused by a
given change in energy retained by the Earth. It makes little
difference whether the change is caused by in increase in solar
intensity or by increasing greenhouse gas levels. This energy, called
radiative forcing, measured in watts per square meter (W/m2). The direct climate
sensitivity (before any feedbacks) is
0.3°
C per W/m2.
However, any change in radiative forcing, from greenhouse gases or
anything else, is amplified by feedbacks. The main positive
feedback is an increase in water vapor, which itself is a greenhouse
gas that causes further warming. This is estimated to be between 1.5 and 2.0 W/m2 per degree K of warming, roughly doubling the the amount of warming that would otherwise occur [Science Feb 2009]. Other positive and negative
feedbacks are described below.
A common defintion of climate sensitivity is the equilibrium global
warming expected to result from doubling the amount of CO2
in the air. The radiative
forcing caused by a doubling of CO2
is well known from atmospheric physics, which is 3.7 W/m2. See The Greenhouse Effect
for more details. The actual (after feedbacks) climate sensitivity can
be determined from
climate models, and from evidence of past climate changes. There
is much more uncertainty than for the direct effects. The most
common estimate is around 3°C for a doubling of carbon dioxide. 3° /
3.7 W/m2
gives a sensitivity of 0.8°
C per W/m2.
Notes:
- Water vapor feedback could amplify the temperature change
due to a doubling of carbon dioxide by some 60%. [ref]
- The current crop of models studied by the IPCC
range from an
equilibrium sensitivity of about 1.5°C at the low end to
4.5°C at
the high end. Differences in cloud feedbacks remain the principal
source of uncertainty.
- From the last glacial to interglacial
transition the
climate sensitivity is approximately 5 oC / 7.1
W
m-2 = 0.7 oC /(W/m2). This
is somewhat higher than that estimated taking into account the
Stefan-Boltzmann response and the water vapour feedback and implies
that there are further feedbacks of importance. Climate models
put the climate sensitivity at 0.3 -
1.4 oC /(W/m2). The uncertainty
arises
mainly from the different treatments of clouds. [ref]
See also [Annan
2005]. The direct sensitivity is calculated here
from Stefan-Boltzmann as 0.26°
C per W/m2.
- The temperature change between full glacial and
interglacial conditions
is about 10ºC in Antarctica, about 3ºC at the Pacific
Warm
Pool on the equator, and 5±1ºC on global average.
We
know the change of surface conditions on the planet quite well, the ice
sheet area being the dominant change. The total forcing of about
6½ W/m2
implies a climate sensitivity of ¾ ±
¼ ºC per W/m2
or 3 ± 1 ºC for doubled CO2. [ref]
- Sydney Levitus of the National Oceanic and Atmospheric
Administration
has analyzed ocean temperature changes of the past 50 years, finding
that the world ocean heat content increased about 10 watt-years per
square meter in the past 50 years. He also finds that the rate of ocean
heat storage in recent years is consistent with our estimate that the
earth is now out of energy balance by 0.5 to one watt per square meter.
That much more solar
radiation
is being absorbed by the earth than is being emitted as heat to
space. Even if atmospheric composition does not change
further,
the
earth’s surface will therefore eventually warm another 0.4 to
0.7
degree C.
Note that the amount of heat required to melt enough ice to raise sea
level one meter is about 12 watt-years (averaged over the planet),
energy that could be accumulated in 12 years if the planet is out of
balance by one watt per square meter. [ref]
A Summary of Positive and Negative Feedbacks
Positive feedbacks:
- The ocean's ability to
dissolve CO2
decreases with
increasing temperature, meaning at the climate warms the ocean will
absorb less CO2
from the atmosphere.
- The atmosphere's ability to
hold water vapor, a greenhouse gas,
increases exponentially with
its temperature. The water holding capacity of the
atmosphere goes up at
about 4% per degree Fahrenheit increase in temperature. A
seven-degree (F) increase in temperature increases water vapor by 25
percent.
- Ice cover decreases with
increasing temperature, decreasing
Earth's
albedo, which will cause more energy to be absorbed.
- Warming ocean surface water at high latitudes may decrease
deep
water circulation, reducing the ability of the ocean to
absorb CO2.
- As the tundra melts, methane may be released from
previously frozen bogs and swamps, causing more greenhouse warming.
Negative feedbacks:
- The greenhouse response of a gas only increases
logarithmically
with its concentration.
- Increased water vapor will
lead to more cloud formation, which
have a
net negative radiative effect.
- Warming in the Arctic will cause more fresh water to enter
the
North Atlantic ocean, which may lead to a weakening in the ocean
current that brings warmth to northern Europe. This may lead
to
local cooling and more ice build up, reversing some of the ice-albedo
effect.
- Warming of the ocean surface may lead to more intense
hurricanes,
the turbulence of which increases deep water circulation, which will
increase the ability of the ocean to absorb CO2.
- Higher concentrations of CO2 cause
plants to grow
faster, which remove CO2 from
the atmosphere.
- A warmer climate can support more vegetation, which will
also remove CO2 from
the atmosphere.
- Higher temperatures and more
rainfall increase erosion, which
(in the long term) removes CO2.
Arctic
Amplification of Greenhouse warming (see also Ice Sheets and Sea Level)
- Ice albedo feedback.
- Arctic is dryer, so less energy goes into evaporation.
- The depth of the atmospheric layer that has to warm in
order to
cause warming of the near-surface air is shallower in the Arctic than
in the tropics.
- Sea ice acts as an insulator. With reduced sea
ice, energy absorbed in summer is more
easily transferred to the air in winter.
There are both positive and negative feedback processes in the
Arctic, occurring over a range of timescales. Positive
feedbacks
include snow and ice albedo feedback; reduction in the
duration of
time that sea ice insulates the atmosphere from the Arctic Ocean; and
permafrost–methane hydrate feedbacks. Negative feedbacks can
result
from increased freshwater input from arctic watersheds, which makes the
upper ocean more stably stratified and hence reduces temperature
increases near the air–sea interface; reductions in the
intensity of
the thermohaline circulation that brings heat to the Arctic; and a
possible vegetation–carbon dioxide (CO2)
feedback that has
the
potential to promote vegetation growth, resulting in a reduced albedo
due to more vegetation covering the tundra.
Probably as a result of natural variations, the Arctic may have been as
warm in the 1930s as in the 1990s, although the spatial pattern of the
warming was quite different and may have been primarily an artifact of
the station distribution.
One might at first suppose that since the amount of water ascending
into clouds increases, the amount of rain that falls out of them must
increase in proportion. But condensing water vapor heats the
atmosphere, and in the grand scheme of things, this must be compensated
by radiative heat loss. On the other hand, simple calculations show
that the amount of radiative heat loss increases only very slowly with
temperature, so that the total heating by condensation must increase
slowly as well. Models resolve this conundrum by making it rain harder
in places that are already wet and at the same time increasing the
intensity, duration, or geographical extent of droughts. Thus, the twin
perils of flood and drought actually both increase substantially in a
warmer world. [ref]
Water Vapor
Water vapor is the main major player in the Earth’s energy
budget, but its concentration in the atmosphere is buffered on a time
scale of weeks by the huge oceanic reservoir of water, which can
rapidly evaporate into the atmosphere and equally rapidly rain out.
Water vapor thus adjusts in response to other changes in climate [ref]
- The total
net absorption [of infrared radiation] over the
whole globe by greenhouse gases is about 75 × 1015
W, an average of 150 W/m2,
roughly one-third by CO2 and two-thirds by water
vapor. [ref]
- Moisture
in the air increases by about 6 percent with every degree Celsius
increase in air temperature. Saturation vapor pressure
increases
rapidly with
temperature; the value
at 90° F (32° C) is about double the value at
70° F (21° C).
- Water vapor greenhouse intensity = 14.817 ln (Wc)
– 4.7318, where Wc = water content of the atmosphere in 1012
tons. The water vapor content of the atmosphere varies
between 12 and 13.5 x 1012
tons (or 15.5 according to the chart below). [ref]
- Due to the changing partial pressure of water vapor in air
as
temperature changes, the water content of air at sea level
can get as high as 3% at 30° C (86° F), and no more
than about 0.5% at 0 °C (32 °F).
- If all the water vapor in the air at a particular time were
to condense
and fall as rain, it would amount to a depth of only about 2.5 cm. This
is called precipitable water. Because water vapor is not evenly
distributed globally, there would be about 5 cm near the equator and
less than one tenth as much at the poles. [ref]
- Water vapor decreases rapidly with height as the atmosphere
gets
colder. Nearly half the total water in the air is between sea level and
about 1.5 km above sea level. Less than 5-6% of the water is above 5
km, and less than 1% is in the stratosphere, nominally above 12 km.
Relative humidity also tends to decrease with height, from
an average value of about 60-80% at the surface to 20-40% at 300 mbar
(9 km). Despite the small amount of water vapor in the upper
troposphere (above 5 km) and stratosphere, recent research has
shown that upper tropospheric water vapor is very important to the
climate. [ref]
- At the prevaling relative humidity in the tropics of 80% at
28°C the water vapour volume mixing ratio is 3%. The other
extreme is the tropical tropopause which acts as a cold trap. For a
tropical tropopause at 16 km and a temperature of
-80°C the water vapour saturation mixing rattio is only
5.5 ppm. [ref]
- It is found that in the case of the increase of the water
content by
10%, the greenhouse intensity would be 34.3 °C, so the change
is
1.34 °C compared to the original value. It means that a higher
or
more intensive greenhouse effect can be expected than it is at present.
Thus, if the water vapour content decreases by 10%, the greenhouse
effect decreases by 1.6 °C. [ref]
- In
the
atmosphere, the molar concentration of CO2
is in the range of 350–400 ppm. Water, on the other hand, has
a very
large variation but, using the “60/60” (60%
relative humidity [RH] at
60 °F) value as an average, then from the American Society of
Heating,
Refrigerating and Air-Conditioning Engineers standard psychrometric
chart, the weight ratio of water to (dry) air is ~0.0065, or roughly
10,500 ppm. Compared with CO2,
this puts water,
on average, at 25–30 times the (molar) concentration of the CO2,
but it can range from a 1:1 ratio to >100:1. [ref]
- An instantaneous forcing calculation for 1.4xH2O
over the whole globe gives a forcing of 5.5 W/m2
[ref
#23]
- Each doubling of water vapor reduces outgoing longwave
radiation (OLR)
by about 6 W/m2
[Pierrehumbert (1999)]. This is about 50% greater than the sensitivity
of OLR to CO2.
[ref] This
means if you take the present water vapor concentration in "typical"
midlatitude sounding, and doubled it or halved it at each level, you
change the OLR by about 6 W/m2
as opposed to 4 W/m2.
You can not use
that figure to extrapolate back to zero water vapor and thus get the
total effect of the present amount of water vapor in the atmosphere.
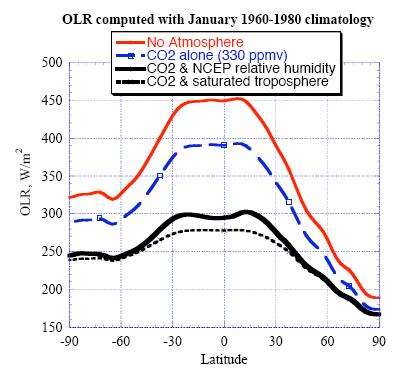
The uneven distribution of water vapor increases its greenhouse effect.
Having some very dry air and some very
moist air allows more infrared
cooling than would the same amount of water spread uniformly over the
atmosphere. To provide some idea of the magnitude of this effect,
consider an atmosphere with a horizontally uniform relative humidity of
50%. Next, increase the humidity in one half of the atmosphere to
87.5%, while keeping the total water in the system constant. This
requires a reduction of the rest of the atmosphere to 12.5% relative
humidity. One not-quite doubles the humidity in half of the atmosphere,
while reducing the humidity in the rest by a factor of 1/4, yielding a
net increase of OLR of 3.6 W/m
2,
based on the sensitivity factor given above. If we make the dry air
still drier,
reducing it to 6.25% while increasing the moist air to 93.75%, the OLR
increase relative to the uniform state becomes 11 W/m
2. [
ref]
Greenhouse
gases high in the atmosphere contribute more to the greenhouse effect.
Any
greenhouse gas, placed near the surface has little effect on OLR,
because the low level air temperature is not much lower than the
surface temperature. To get a significant greenhouse effect, one must
increase the infrared opacity of a portion of the atmosphere that is
significantly colder than the surface. Because the
radiative effect
of water vapor is logarithmic in its concentration, small quantities of
water vapor can accomplish this task aloft. This leads to the concept
of ”Free Tropospheric Humidity,” (FTH) or
”Upper Tropospheric Humidity”
(UTH) which may be loosely defined as the water content of the portion
of the atmosphere where water vapor has a considerable effect on the
radiation budget. Diversion of a tiny proportion of the
atmosphere’s
net water vapor content would be sufficient to saturate the mid to
upper troposphere and radically warm the climate. For example, consider
a 50mb thick layer of saturated air near the surface of the tropical
ocean, having a temperature of 295K. Less than 3% of the water content
of this layer would suffice to completely saturate a layer of equal
thickness having a temperature of 250K, such as would be encountered in
the tropical mid-troposphere, or at lower altitudes in the
extratropics. Clearly, the magnitude of the boundary layer water vapor
reservoir is not the limiting factor in determining the free
tropospheric humidity.
Water vapor
feedback does not contribute to polar amplification.
It
is important to note that water vapor feedback does not contribute to
polar amplification. In fact, for the present pole to equator
temperature range, water vapor feedback makes the slope larger at cold
temperatures than at warm temperatures [Pierrehumbert (2002)],
and hence would lead to tropical rather than polar amplification.
Certainly, ice albedo feedback plays a role in polar amplification, but
dynamical heat transport and clouds may also contribute. These
feedbacks must be sufficiently strong to overcome the tendency of water
vapor feedback to put the greatest warming in the tropics.
The greenhouse
effect is stronger in the tropics.
As
compared to the calculation with no atmospheric greenhouse effect
whatsoever, CO2 by itself
brings the tropical OLR down by 60 W/m2. The OLR reduction
decreases with latitude in the extratropics, falling to
25 W/m2 at 60S in the summer
hemisphere, and even smaller values in the winter extratropics. The
latitudinal variation in the CO2 greenhouse effect
derives from the vertical structure of the atmosphere: In the tropics
there is more contrast between surface and tropopause temperature than
there is in the extratropics, and the summer extratropics has more
contrast than the winter extratropics. When OLR is recalculated with
the observed humidity content of the atmosphere (based on NCEP) in
addition to the CO2 from the previous
case, OLR drops by an additional 100 W/m2 in the tropics, and a
lesser amount in the extratropics. In fact, at each latitude the
greenhouse effect of water vapor is approximately twice that
of CO2.
Clouds, Aerosols and Albedo
Clouds are the least understood part of the climate system.
Cloud cover reduces ground-level radiation by a global
average of about 30 W/m2
[ref].
They
have a cooling effect by reflecting visible light from the sun, and a
warming effect by absorbing infrared energy from the Earth's surface.
High altitude cirrus clouds are largely transparent to light,
but
are more effective at absorbing infrared energy because they are
cooler. Low altitude clouds have less of an infrared effect
because they are warm, but a large change in albedo because they are
thick and opaque. Clouds are believed to have a net cooling
effect. As cloud levels increase with higher water vapor
levels,
they are expected to act as a negative feedback to greenhouse warming.
Notes on cloud effects on climate:<
- Clouds don't invariably cause cooling during the
day
because clouds can
have a strong greenhouse effect -- and you've got no cause to ignore
the night-time half of the equation. Increased water vapor does not
lead to increased clouds, since increased temperature can dissipate
clouds. Clouds are not simply related either to water vapor content or
to temperature. [ref]
- A CO2-induced
increase in low clouds
mainly
acts to reflect more solar radiation and thus would provide a negative
feedback to global warming. An increase in high clouds mainly adds to
the
absorption of infrared radiation trying to escape the planet and would
thus provide a positive feedback. A change in cloud microphysical and
optical
properties could go either way. [ref]
- A change in cloud droplet size from 10
microns
down to 8
microns
has the same effect on the radiation balance of the earth as doubling
the CO2 [But
what exactly is going to cause such a change?]
- Over the last 15 years, without anyone’s
knowledge, the
amount of
thermal, long-wave radiation escaping the atmosphere above the tropics
increased by 4 watts per square meter. At the same time the amount of
reflected sunlight, which is mostly in the form of short-wave visible
and near-visible light, decreased by 4 watts per square meter.
Human-generated greenhouse gases have thus far led to a
0.5-watts-per-square-meter increase in the solar energy absorbed into
the atmosphere, while the tropical radiation changes due to cloud cover
were almost ten
times as large. [ref]
- Different types of clouds have different
albedo values, theoretically ranging from a minimum of near 0% to a
maximum in the high 70s. Climate models have shown that if
the whole earth were to be suddenly covered by white clouds, the
surface temperatures would drop to a value of about -151°C
(-240°F). This model, though it is far from perfect, also
predicts
that
to offset a 5.0°C (9°F) temperature change due to an
increase in the magnitude of the greenhouse effect, "all" we would need
to do is increase the earth's overall albedo by about 12%
by adding more white clouds. [ref]
Aerosols
Aerosols are solid or liquid particles suspended in the
atmosphere,
consisting of (in rough order of abundance): sea salt, mineral dust,
inorganic salts such as ammonium sulfate (which has natural as well as
anthropogenic sources from e.g. coal burning), and carbonaceous aerosol
such as soot, plant emissions, and incompletely combusted fossil fuel.
As should be apparent from this list, there are many natural sources of
aerosol, but changes have been observed in particular, in the
atmospheric loading of carbonaceous aerosol and sulphates, which
originate in part from fossil fuel burning. While a relatively minor
part of the overall aerosol mass, changes in the anthropogenic portion
of aerosols since 1750 have resulted in a globally averaged net
radiative forcing of roughly -1.2 W/m2, in
comparison to the overall average CO2 forcing of
+1.66 W/m2. [ref]
Fossil fuel burning generates both aerosols and carbon dioxide, which
act as cooling and warming forcings respectively. The amount of
aerosols depends greatly on what fuel is being used. However, the
cooling effect of the aerosols can be a substantial fraction of the
warming effect of the carbon dioxide. If we were to remove, for
example, a coal fired generating station, the aerosol cooling effect
would end in a week, while it would take some time before effects of
the carbon dioxide to be felt. That means closing the coal plant
actually causes global warming for a while, a period that could be as
much as twenty years.
Let me attempt a crude calculation of how long it takes before a
reduction in fossil fuel use lead to cooling. I will start by assuming
all aerosols and carbon dioxide come from fossil fuel burning (in
reality it is only part of both, so maybe the errors roughly cancel).
According to figure SPM-2 (shown above),
stopping
fossil fuel use would result in an immediate increase in forcing of 1.2
watts per square meter (W/m2), the sum of the
two aerosol forcings. The present forcing from carbon dioxide is 1.7 W/m2.
Assuming the relationship between CO2
concentration and forcing is linear (not true: it is logarithmic, each
unit of decrease will have more effect, but that is good enough for
this crude calculation) we need to get rid of 70% of the CO2
to remove 1.2 W/m2 of forcing.
Reading from the CO2 decay
rate from this
page (also shown below),
it will take one hundred years to reduce CO2
by 70%. However the result is very sensitive - if we assume we only
need a 50% CO2
reduction (because of the large uncertainty in aerosol forcing or other
problems with the figures I use), the time is reduced to 20 years.
Even
the more optimistic result indicates we get net warming for 20 years
after reducing fossil fuel use. I wonder how many people are really
aware of the long time scales involved in this issue. There are no
quick fixes. This is not a justification for doing nothing, but we must
be realistic.
Albedo
Albedo is a measure of how much light is reflected from the
Earth.
The greater the albedo, the more light is reflected, and the
cooler the planet. The average albedo of the Earth is about 30%,
but varies seasonally:
Winter: 0.309, Spring: 0.2906, Summer: 0.2878, Autumn: 0.310.
Changing albedo through land use changes can affect the climate.
The Earth's Albedo in
March 2005
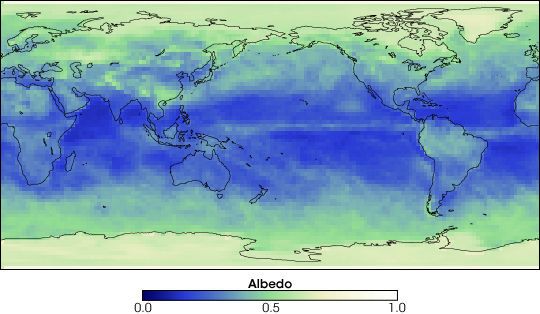
Albedo Notes:
- A 1% change in the Earth's albedo (as opposed to
changing
albedo by
0.01) results in a 1 W/m2 change
in radiative forcing, so it would take almost 4% to be equivalent to
doubling of
atmospheric CO2.
A drop of as little as 0.01 in Earth’s albedo
would have a
major
warming influence on climate—roughly equal to the effect of
doubling
the amount of carbon dioxide in the atmosphere, which would cause Earth
to retain an additional 3.4 watts of energy for every square meter of
surface area. [ref]
- When changing from grass and croplands to forest,
there are
two
competing effects of land cover change on climate: an albedo effect
which leads to warming and an evapotranspiration effect which tends to
produce cooling. It is not clear which effect would dominate. We have
performed simulations of global land cover change using the NCAR CAM3
atmospheric general circulation model coupled to a slab ocean model. We
find that global replacement of current vegetation by trees would lead
to a global mean warming of 1.3°C, nearly 60% of the warming
produced under a doubled CO2 concentration, while replacement by
grasslands would result in a cooling of 0.4°C. It has been
previously shown that boreal forestation can lead to warming; our
simulations indicate that mid-latitude forestation also could lead to
warming. These results suggest that more research is necessary before
forest carbon storage should be deployed as a mitigation strategy for
global warming. [ref]
- Ocean surface albedo is highly variable and is
sensitive to
four
physical parameters: solar zenith angle, wind speed, transmission by
atmospheric cloud/aerosol, and ocean chlorophyll concentration [ref].
Wind speed has small effect on albedo at high sun, but its
effect
increases as Solar Zenith Angle increases. Wind affects Ocean Solar
Absorption mainly by changing the
slopes of wave facets.. Aerosols scatter light, which
therefore
scatters the incident angles as well. Ocean albedo can vary
from
0.03 to 0.1, and up to 0.4 for calm water at low sun angles.
Variations in Solar Energy Reaching the Earth
The climate system is almost entirely driven by energy from
the sun.
The sun's influence on climate change is determined by variations in
solar output. This has been accurately measured by satellites over the
past few decades, as shown below. The dominant feature is the 11 year
sunspot cycle, which causes a change of 0.08%.
The total insolation above the Earth is about 1,365 W/m2. Because the Earth is
round, most of the radiation reaches the Earth's
surface obliquely, and half of the Earth is in total darkness, so this
figure must be divided by four, which gives 341 W/m2. Then multiply
by 0.7 to take into account the portion that is reflected back into
space (albedo), which gives 239 W/m2.
Therefore the sunspot cycle variation amounts to about 0.17 W/m2. This amount of change
is hard to detect in the climate record (but see [ref])
because it is smaller than the annual temperature variation from other
causes. This is the equivalent direct forcing as adding seven years
worth of carbon dioxide to that atmosphere at the current rate of 2 ppm
per year.
 |
Percentage change in monthly values of the total solar
irradiance composites of Willson and Mordvinov (2003; WM2003, violet
symbols and line) and Fröhlich and Lean (2004; FL2004, green solid
line).
Source: IPCC
AR4 WG1, ch 02, fig. 2.16 |
Measuring change in solar irradiance over the past few centuries
requires the use of indirect records. There is a good record of the
change in sunspot number. Low numbers of sunspots correspond to lower
insolation. Knowledge of solar activity is also inferred indirectly
from the 14C and 10Be
cosmogenic isotope records in tree rings and ice cores. These measures
clearly show the sunspot cycle, but determining if there is a change in
the overall magnitude of solar output over this period is more
difficult. This can be determined by observing changes in stars that a
similar to the Sun. Recent information shows that solar variation is
smaller than was once thought. The IPCC 2007 report claims the change
in solar irradiance since 1750 is
estimated to cause a radiative forcing of +0.12 [+0.06 to
+0.30] W/m2,
which is less than half the estimate given in IPCC 2001.
IPCC 2007 states that the increase in total irradiance from the Maunder
Minimum (a period in the 17th century with low sunspot activity) to
current cycle minima is 0.04% (an irradiance increase of roughly
0.5 W/m2
in 1,365 W/m2),
corresponding to a forcing of +0.1 W/m2.
 |
Reconstructions of the total solar irradiance time
series starting as
early as 1600. The upper envelope of the shaded regions shows
irradiance variations arising from the 11-year activity cycle. The
lower envelope is the total irradiance reconstructed by Lean (2000), in
which the long-term trend was inferred from brightness changes in
Sun-like stars. In comparison, the recent reconstruction of Y. Wang et
al. (2005) is based on solar considerations alone, using a flux
transport model to simulate the long-term evolution of the closed flux
that generates bright faculae.
Source: IPCC
AR4 WG1, ch 02, fig. 2.17 |
Compare this to the paleoclimate
data for the past 1000 years.
Carbon
Dioxide and Methane
Carbon
dioxide levels in the atmosphere have risen from
270 ppm to 380 ppm, or by
roughly 25%.
We know that almost all of that increase is human caused
for several reasons. Humans have produced twice as much
carbon
dioxide than the amount that has been added to the atmosphere.
We
are currently adding 2.8 ppm of CO2
to the atmosphere each year, but the amount that remains in the air is
only
increasing by nearly 2 ppm per year.
The rest is going into land vegetation and the
ocean. The
only possible natural source for carbon dioxide is the ocean, which
contains 50 times more dissolved CO2
than the atmosphere. But if CO2
is going into the ocean it cannot also be coming out the ocean. The CO2
concentration in the ocean has been measured
over time, and has been found to be increasing. The
atmosphere
contains carbon-14 caused by cosmic ray bombardment of nitrogen.
Fossil fuels contain no carbon-14 because it has all decayed.
Carbon-14 levels in the atmosphere have been decreasing
because
they have been diluted with carbon from fossil fuels. See [How Do We Know
that the Atmospheric Build-up of Greenhouse Gases Is Due to Human
Activity?]
How long does added
carbon dioxide stay in the air?
Most
of the
carbon dioxide that we add to the atmosphere will be
absorbed by the ocean.
The lifetime of carbon dioxide in the atmosphere is often
mistakenly quoted as being on the order of a hundred years; this figure
is actually the result of a fallacious and largely meaningless method
of aggregating the many physical processes that operate on widely
differing time scales into a single number which is supposed to
represent the amount of time some extra added carbon dioxide will stay
in the atmosphere. The fact is that for each kilogram of carbon dioxide
put into the atmosphere today, only a small portion will be rapidly
absorbed into the ocean. After five hundred to one thousand years of
slow uptake by the ocean, fully a quarter of that kilogram will remain
in the atmosphere. A portion of that will be taken up by the ocean over
the next ten thousand years by slow processes related to ocean
sediments, but fully 7 percent of our initial kilogram will stick
around for hundreds of thousands of years. [ref]
Global Warming Potential and the Relative Effect of
Carbon
Dioxide and Methane on the Greenhouse Effect
Global Warming
Potential
(GWP)
is a term used to compare the relative effect of greenhouse
gases. For example, methane is said to be 22 times more potent
than
carbon dioxide. However, this value depends on the
concentration of that gas in the atmosphere, and its atmospheric
lifetime.
The
common statement that methane is, molecule for molecule, a
better greenhouse gas than CO2
is true only for situations like the
present where methane is present in far lower concentrations
than CO2.
In this situation, the greater power of a molecule of CH4
to
reduce the OLR (outgoing longwave radiation) results simply from the
fact that the greenhouse effect
of both CH4
and CO2
are approximately logarithmic in concentration. For methane
concentrations of
around 1 ppmv, each doubling of methane reduces OLR by about
2 W/m2.
On
the other hand, for CO2
concentrations near 300 ppmv, each doubling of CO2 reduces the OLR by
about 6 W/m2.
Hence, to achieve the same OLR reduction as a doubling of CO2 one needs three
doublings of methane, but since methane starts from a concentration of
only 1 ppmv, this only takes the concentration to 8 ppmv, and requires
only 7/300 as many molecules to bring about as was
needed to achieve the same reduction using a doubling of CO2.
Equivalently, we can say that adding 1 ppmv of methane yields as much
reduction of OLR as adding 75 ppmv of CO2. If methane were the
most abundant long-lived greenhouse gas in our atmosphere,
and CO2
were present only in very small concentrations,
we would say instead that CO2
is, molecule for molecule, the better
greenhouse gas. [ref]
The table below shows the predicted rate at which carbon dioxide
released to the atmosphere is absorbed. In 20 years almost half of
it is gone, in 100 years 25% of it still remains, and in 100,000 years
7% is still there.[ref]
The ocean absorbs CO2
until the partial pressure in the ocean reaches the new
level that is in the air. After that, CO2
is removed by the silicate weathering cycle over a timescale of 400,000
years. This is true for present levels of carbon dioxide;
at higher
levels the ability of the environment to absorb CO2 is
reduced. However, that has not changed in the last fifty years, as seen
here.
The atmospheric
concentration of carbon dioxide appears to
be increasing exponentially since 1750 [ref] |
|
Accurate
measurements at Mauna
Loa (in Hawaii) over
the last fifty years
show what looks to be a linear increase.. |
 |
|
 |
...but the rate of increase is gradually rising, reaching 2 ppm per
year.
|
The
Direct Health Effects of Carbon Dioxide
Health
effect
on humans due to CO2
levels start at around 1% (10,000 ppm). For example, this
source states: "The
Occupational Safety and Health Administration (OSHA) and the American
Conference of Governmental Industrial Hygienists (ACGIH) have set
workplace safety standards of 5,000 ppm as an 8-hour time weighted
average (TLV-TWA) exposure, and 30,000 ppm as the short term exposure
level (STEL)."
Trends in Carbon Dioxide and Methane Concentration
Carbon dioxide is continuing to increase linearly, in line
with IPCC
2001 projections (in yellow). Anthropogenic methane sources, such as
rice paddies, the fossil fuel
industry, and livestock, have already more than doubled the methane
concentration in the atmosphere from pre-industrial
levels. The
atmosphere currently contains about 3.5 Gton C as methane. However,
methane levels have
started to level off, falling far below the projections. Since
1999 there has been essentially no growth in the mean
annual atmospheric methane concentration, compared to a 15%
rise over the preceding 20 years. [ref]
The
cause of this change is unknown, so it may only be temporary.
 |
 |
Recent
CH4 Concentrations and Trends
(a)
Time series of global CH4
abundance mole fraction (in ppb) derived from surface sites operated by
NOAA/GMD
(blue lines) and AGAGE (red lines). The thinner lines show
the CH4
global averages and the thicker lines are the de-seasonalized global
average trends from both networks.
(b)
Annual growth rate (ppb/yr) in global atmospheric CH4
abundance from
1984 through the end of 2005 (NOAA/GMD,
blue), and from 1988 to the end
of 2005 (AGAGE, red).
[From IPCC
AR4 WG1 Ch 2, p 142] |
 |
Residence
Time of Methane in the Atmosphere
The residence time of methane at the present concentrations
is about
eight years. Methane is removed from the air by hydroxyl radicals
(OH-), which are produced by ultraviolet light being absorbed by ozone,
and the decomposition of nitrogen dioxide (NO2).
If you increase
methane levels, the hydroxyl gets used up and the residence time
increases, leading to more greenhouse warming. However, it would take a
tenfold increase in methane levels to double the residence time from 8
to 16 years. |
Methane Hydrates
For most parts of the ocean, melting of hydrates is a slow
process. It
takes decades to centuries to warm up the water 1000 meters down in the
ocean, and centuries more to diffuse that heat down into the sediment
where the base of the stability zone is. The Arctic Ocean may be a
special case, because of the shallower stability zone due to the colder
water column, and because warming is expected to be more intense in
high latitudes. [ref]
Total amounts of methane hydrate in permafrost soils are very poorly
known, with estimates ranging from 7.5 to 400 Gton C.
The Effect of the Ocean on Carbon Dioxide Uptake
and Thermal
Lag
The oceans have absorbed about 48% of all carbon dioxide
produced by
fossil fuels in the 20th century, about 120 Gt in all. Without this
oceanic uptake, atmospheric CO2
would be about 55 ppm higher today than what is currently
observed The figure below shows that this
anthropogenic CO2 is
not evenly distributed throughout the oceans. The highest vertically
integrated concentrations are found in the North Atlantic, due to the
overturning effect of the Gulf Stream. As a result, this ocean basin
stores 23% of the global oceanic anthropogenic CO2,
despite covering only 15% of the global ocean area. By contrast, the
Southern Ocean south of 50°S has very low vertically integrated
anthropogenic CO2
concentrations, containing only 9% of the global inventory [Science
July 2004].

Column inventory of anthropogenic CO2
in the ocean (mol/m2).
High inventories are
associated with deep water formation in the North Atlantic and
intermediate and mode water
formation between 30° and 50°S. Total inventory of
shaded regions is 106 ± 17 Pg C.
While colder water can hold more CO2 than
warmer water, the critical factor for the amount absorbed from the
atmosphere depends on how saturated with CO2 the water already is.

Map of the 1994 distribution of Revelle factor, averaged
for the
upper 50 m of the water column. A high Revelle factor indicates that,
for a given atmospheric CO2 perturbation,
the oceanic equilibrium concentration of anthropogenic CO2
will be lower than that for low–Revelle factor waters. The
current Revelle factors are about one unit higher than they were in the
preindustrial ocean.
There are several reasons
why the ocean may not always continue to abosrb carbon dioxide at the
same rate as the recent past:
1) The solubility of CO2 decreases
with temperature. As the ocean gets warmer, it will be able
to absorb less carbon dioxide, so more will remain in the atmosphere.
For a temperature increase of
1°C,
the partial pressure of CO2
in the ocean surface layer increases by 4.2% or 15 μ atm., which
corresponds to an average net flux of around 4 Gt C to the atmosphere.
This means that a sea surface temperature increase of 1°C
leads to a CO2
flux to the atmosphere that is twice as big
as its current yearly take up of anthropogenic CO2. [ref]
2) The ability of the ocean to absorb CO2
at the same temperature decreases as carbon dioxide levels increase.
If atmospheric CO2
increases 10%, the ocean concentration will rise 1%. This
will occur in the top 50 to 100 meters.
Because an increased atmospheric CO2
content increases the
oceanic carbon dioxide uptake, more carbonate is also used during
chemical solution processes, and there is increasingly less carbonate
available for the chemical reaction with carbon dioxide. Thus an
increasing share of the carbon dioxide taken up remains in its original
form in the water, and the possibility that the surface water takes up
further carbon dioxide from the atmosphere is decreased. This effect is
relatively large: for a further increase of atmospheric CO2
concentrations of 100 ppm (i.e. from 370 to 470 ppm) the CO2
uptake by the ocean is reduced by 40% compared to during the first 100
ppm increase from 280 to 380 ppm since the start of industrialisation. [ref]
3) Rising temperatures cause increased
stratification of the
ocean surface layer. That reduces the rate of transfer of
carbon dioxide to the deep ocean, leaving the upper layer more
saturated, thus less able to absorb CO2.
Mixing by turbulence in the ocean
is
essential for moving CO2
down into
the deep ocean, away from the top 100 meters of the ocean, where carbon
absorption from the atmosphere takes place. With increased
temperatures, the ocean stratifies more, mixing becomes harder,
and CO2
accumulates in the surface ocean instead of in the deep ocean. This
accumulation creates a back pressure, lowering CO2
absorption. [ref]
4) The ocean is absorbing much of the energy from
increased
greenhouse gases. This change in equilibrium cannot continue,
and the heat will eventually be returned to the atmosphere, further
increasing warming.
The heat-carrying capacity of the
global
ocean is over 1000 times
greater than that of the global atmosphere. The current
unrealised warming "in the ocean pipeline" is related to the net
imbalance, 0.85 ± 0.15 W/m2
implies an further warming of around 0.5-0.7°
C, regardless of future emission increases. [So why did 2.5 W/m2 of greenhouse gas
forcing cause 0.8°
C in the 20th century, but 0.85 W/m2 cause 0.7°
C?]
5) Rising ocean temperatures may reduce the ability
of
phytoplankton to remove carbon dioxide.
Diatoms are the most important
group of
phytoplankton for
removing carbon from the atmosphere and it's the silicon in diatoms
that makes
carbon removal possible. Temperature dramatically influences
how well diatoms can do this. A coating of
carbon surrounds and protects silicon in diatoms. In cold water, like
that found near Antarctica, slower bacterial action allows more carbon
to remain attached to the silicon. As the diatoms sink in the cold
water, they take the carbon with them to the ocean bottom. The carbon
can remain there for thousands of years away from the atmosphere. Even
small increases in temperature cause bacteria to quickly eat the
coating. The diatoms dissolve more readily and carbon recycles back to
the surface ocean instead of being sequestered in deep waters.
Thus,
the warmer the ocean, the less able it is to pull carbon
out of the atmosphere. [ref]
6) Global warming will reduce the temperature
difference
between
the polar and equatorial regions. This temperature difference is the
driving force for most of the world's weather. Reducing the driving
force may reduce the intensity of winds, which are responsible for
causing mixing of the ocean's layers. This may lead to increased
startification, thus reduced carbon dioxide uptake, and reduced
oxygenation of the deep ocean.
On the other hand, this summary of a Lawrence
Livermore National Laboratory study states "The model shows that ocean uptake of CO2
begins to decrease in the 22nd
and 23rd centuries due to the warming of the ocean surface". This model
is based on a rather high emissions scenaro lasting 300 years,
predicting 8°
C of warming. But change in
ocean uptake does not kick in for a century. It also says "the
most drastic changes during the 300-year period
would be during the 22nd century in which precipitation change, an
increase in atmospheric precipitable water and a decrease in sea ice
size are the largest when emissions rates are the highest. During the
model runs, sea ice cover disappears almost completely in the northern
hemisphere by the year 2150 during northern hemisphere summers."
[In
2008, the extent of Arctic sea ice is decreasing rapidly, and now it
appears that the Arctic will be ice free in summer before 2020.]
Warmer water contains less oxygen, as shown below:

The ratio of annual atmospheric CO2
increase to annual fossil fuel CO2
emissions has remained constant since 1950. For up to date
information on CO2
emissions, see the Mauna Loa
Observatory.

Notes:
- A mean temperature change of 0.1°
C of the world ocean would correspond roughly to a mean temperature
change of 100°
C of the global atmosphere if all the heat associated with this ocean
anomaly was instantaneously transferred from the ocean to the
atmosphere. This of course will not happen but this
computation
illustrates the enormous heat capacity of the ocean versus the
atmosphere. [ref]
[But
only the top layer of the ocean interracts with the atmosphere in the
short term. If we assume the top layer is 100 m deep, and the
oceans have an average depth of 4 km, only 0.025% of the ocean matters.
So effectively, the heat capacity of the top layer of the
ocean
is only 25 times that of the global atmosphere]
- Solar heating of the ocean on a global average is
168 watts
per square
meter... The ocean transmits electromagnetic radiation into the
atmosphere in proportion to the fourth power of the sea surface
temperature (°K)... Net back radiation cools the ocean, on a
global
average by 66 watts per square meter.
- When air is contact with the ocean is at a
different
temperature than
that the sea surface, heat transfer by conduction takes place. The
ocean is on global average about 1 or 2 degrees warmer than the
atmosphere so on average ocean heat is transferred from ocean to
atmosphere by conduction... On global average the oceanic heat loss by
conduction is only 24 watts per square meter... On global
average
the heat loss by evaporation from the ocean is 78 watts per square
meter. [ref]
- Saltier water can hold less carbon dioxide (6.5
ppm less
for a 3% increase in salt content).
- Cooling of sea water reduces pCO2
by 4.1–4.25% per °C, marine
phytoplankton blooms reduce both pCO2
and total CO2
(refs 7, 8), while mixing upwards of CO2 from CO2-rich subsurface water
tends to increase surface pCO2.
- Surface pCO2
ranged from 400 to 420 µatm
- Annual changes were typically 80–100
µatm
- A cooling of 4 °C should
result
in a decrease of pCO2
by 50–55 µatm.
- We assumed that seawater pCO2
remained at 410 µatm until the height of the storm, and then
declined linearly to 360 µatm at the end of the storm. To
calculate CO2
fluxes, we assumed that delta pCO2
values were +65 µatm at the beginning of the storm,
+15 µatm
at the end atmospheric pCO2
decreased by 20 µatm in response to a transient 6%
drop in atmospheric pressure (from 1,020 to 965 mbar).
- Hurricanes and tropical storms in the latitudinal
band
40° S
to 40°N should contribute to the ocean-to-atmospheric flux
of CO2
by between +0.042 and +0.509 Pg C / yr
- Although we expect that changes in
surface-to-deep mixing
remains the primary control of ocean uptake of CO2 over multi-year,
decadal timescales, CO2
fluxes due to hurricanes provide an additional secondary feedback
mechanism that is not accounted for in present global carbon cycle and
climate models.
The increase in heat content from 1957 to 1994, the period of best data
coverage, is 19.0 (± 9.0) x 1022
J. The heat content increase yields 0.32 ±
0.15 W/m2
(expressed per unit area of the entire world, not just the ocean
surface). [ref]
Atmospheric CO2 and Acidification of the Ocean
Surface oceans have an average pH globally of about 8.2
units. However,
pH can vary by ±0.3 units due to local, regional and
seasonal
factors. [ref]
Only the near-surface waters, or surface layers, of the oceans (down to
about 100 m on average) are well mixed and so in close contact with the
atmosphere. Carbon dioxide in the atmosphere dissolves in the surface
waters of the oceans and establishes a concentration in equilibrium
with that of the atmosphere. Molecules of CO2
exchange readily with the atmosphere and on average only remain in the
surface waters for about 6 years. However mixing and advection
(vertical motions, sinking and upwelling) with the intermediate and
deep waters of the oceans (down to about 1000 m and 4000 m
respectively) is much slower, and takes place on timescales of several
hundred years or more.
The fastest natural changes that we are sure about are those occurring
at the ends of the recent ice ages, when CO2
rose about 80 ppm in the space of 6000 years (IPCC 2001). This rate is
about one-hundredth that of the changes currently occurring. During
slow natural changes, the carbon system in the oceans has time to
interact with sediments and stays therefore approximately in steady
state with them.
Although the biological uptake of CO2
per unit area of the surface
oceans is lower than that in most terrestrial systems, the overall
biological absorption is almost as large as that in terrestrial
environment. This is because the surface area of the oceans is so much
larger. Increasing CO2 in
ocean water will increase productivity of photosynthesis by less than
10%, because even at today’s CO2
concentration photosynthesis is saturated with inorganic carbon.
Ocean pH has fallen by 0.1 units in the 20th century. If
global emissions of CO2
from human activities continue to rise on
current trends then the average pH of the oceans could fall by 0.5
units (equivalent to a three fold increase in the concentration of
hydrogen ions) by the year 2100 [based
on a very high emissions scenario - CO2
reaching 2000 ppm by the year 2200, compared
with 380 ppm today]. This pH is probably lower than has
been experienced for hundreds of millennia and, critically, this rate
of change is probably one hundred times greater than at any time over
this period. The scale of the changes may vary regionally, which will
affect the magnitude of the biological effects.
As atmospheric CO2
levels increase so does the concentration of CO2
in
the surface oceans. However it is unlikely that the past atmospheric
concentrations would have led to a significantly lower pH in the
oceans, as the rate at which atmospheric CO2
changed in the past was
much slower compared with the modern day. The fastest natural changes
that we are sure about are those occurring at the ends of the recent
ice ages, when CO2
rose about 80 ppm in the space of 6000 years (IPCC
2001). This rate is about one-hundredth that of the changes
currently occurring. During slow natural changes, the carbon system in
the oceans has time to interact with sediments and stays therefore
approximately in steady state with them.
Marine organisms that construct CaCO3
structures, such as
shells, are dependent on the presence of bicarbonate and carbonate
forms of dissolved inorganic carbon in seawater. Once
formed, CaCO3
will dissolve back into the water unless the surrounding seawater
contains sufficiently high concentrations of carbonate ions (CO3
2-),
ie. it is saturated. Calcium carbonate also becomes more soluble with
decreasing temperature and increasing pressure, and hence with ocean
depth. A natural boundary develops in seawater as a result of
these different variables. This is known as the ‘saturation
horizon’ and it identifies a clear depth of seawater above
which CaCO3
can form, but below it
dissolves. Increasing CO2
levels and the resultant lower pH of seawater decreases the saturation
state of CaCO3 and
raises the saturation horizon
closer to the surface.
Not all marine organisms respond to increased carbon dioxide levels the
same way. In this Science
paper: From the mid-Mesozoic, coccolithophores have
been major calcium carbonate producers in the
world's oceans, today accounting for about a
third of the total marine CaCO3 production.
Here, we present laboratory evidence that calcification and
net primary production in the coccolithophore species Emiliania
huxleyi are significantly increased by high CO2
partial pressures. Field evidence from the deep
ocean is consistent with these laboratory
conclusions, indicating that over the past 220 years there
has been a 40% increase in average coccolith mass.
The only period with a similar rapid increase of carbon dioxide was the
Paleocene
Eocene Thermal Maximum, which occured 55 million years ago.
Ocean sediments from this time reveal a large die-off of calcium
carbonate based organisms, which is described in this
paper in Science.
A related issue is the removal of oxygen from the oceans caused by
the runoff of organic matter from the land. This leads to regions of
the ocean that are effectively dead. This map
correlates the human footprint with observed dead zones, from [Science
Aug 2008].
Finally, all the proof we ever need...

Return
to the Climate Change Main Page
